The Royal Swedish Academy of Sciences
awarded the 2011 Nobel Prize in Physics to three astronomers for
discovering the accelerating expansion of our universe. This was one of
the most dramatic and surprising discoveries of the 20th century, found
independently by two groups who set out to measure the universe’s deceleration — the exact opposite of what they discovered.
This major discovery seems to settle a long-standing cosmic question: “Will the universe end in fire or ice?” By fire, we mean the universe eventually re-collapsing to a tiny point with an immense temperature. By ice,
we mean the universe continuing to expand forever and becoming ever
colder. If the observed expansion rate continues to accelerate, the
universe will end in ice. Either alternative would play out over hundreds of billions of years. There’s no rush to stock up on arctic clothing.
It’s gratifying that the Nobel committee
has begun recognizing the contribution of observational astronomy. None
of the first 76 Nobel Prizes in Physics went to astronomers, not even
to Edwin Hubble or Henrietta Leavitt. Even now, the total number of
Nobel Prizes awarded to astronomers can be counted on one hand, with
fingers to spare.
Saul Perlmutter of the Lawrence Berkeley
National Laboratory led one group that began its work in 1988. Brian
Schmidt, born and educated in the US and now at the Mount Stromlo
Observatory in Australia, launched a second group in 1994; their data
was mainly analyzed by Adam Riess at UC Berkeley.
The Nobel committee awarded half the
prize money to Perlmutter (about $700,000) and one quarter each to
Schmidt and Riess (about $350,000 each). (The prize money is exempt from
income tax.) About 20 astronomers participated in each group, but Nobel
Prize rules mandate no more than three awardees.
Both groups searched for exploding stars
of a specific kind — type 1a supernova—and measured their distance and
velocity relative to Earth. This allowed them to measure the expansion
rate of the universe at various times during the 13.75 billion years
since the Big Bang. Contrary to virtually every scientist’s
expectations, they found that the expansion rate is now increasing — the expansion is accelerating.
The primary scientific data are shown below (I reorganized and relabeled it to improve clarity):
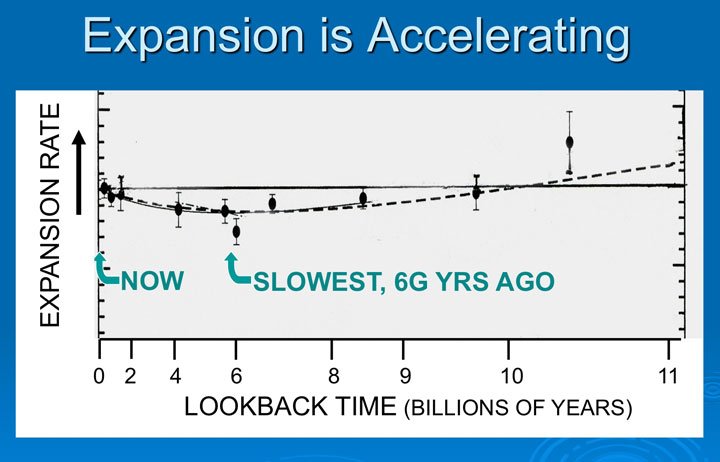
In the above chart, the expansion rate
of the universe (how rapidly the universe is growing) is shown on the
vertical axis — faster expansion is at the top and slower expansion is
at the bottom. Time is shown on the horizontal axis — today is all the
way to the left, and 11 billion years ago is to the right — this is
called “Lookback Time.” The data point with the highest measured
expansion rate is at the upper right, between 10 and 11 billion years
ago. The dots indicate the measured values and the vertical bars denote
the measurement uncertainties. Because supernovae are very far away and
even our best telescopes collect only a modest amount of their light,
measurement precision is limited. Properly determining the uncertainty
in measurements is an essential part of science.
Beginning at the right, the chart shows
what everyone expected — the expansion rate was initially very high and
gradually decreased from 11 billion to 6 billion years ago. This makes
sense. As galaxies fly apart from the Big Bang, each one pulls on all
the others with the force of gravity from the mass of its billions of
stars. As every galaxy pulls on every other one, the expansion rate will
slow down. The dashed curve shows the overall trend of the data. It
clearly decreases from 11 billion years ago to 6 billion years (“6G
YRS”) ago.
The great surprise is what has happened
since 6 billion years ago. The expansion rate reached a minimum at that
time, and has increased ever more rapidly to the present (“NOW”) — the
expansion rate is accelerating.
For the expansion rate to increase,
something must now be pushing the universe apart more forcefully than
the gravity of all the galaxies pulling it together — there must now be a
dominating source of “negative” gravity. Scientists have named this
mysterious phenomenon “dark energy.”
We’ll explore dark energy later. Let’s
talk first about how Perlmutter, Schmidt, Riess, and their colleagues
made their measurements.
Type 1a supernovae are a very special
class of exploding stars. A beautiful example taken by Hubble is shown
below — supernova SN1994D on the outskirts of spiral galaxy NGC 4526.
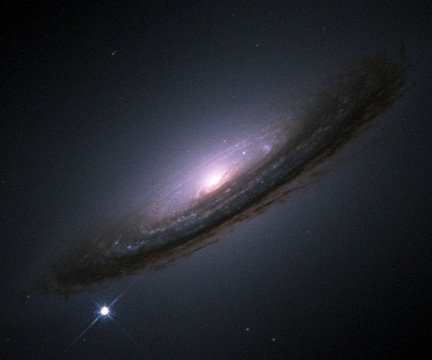
Three characteristics make them
particularly useful for this project. Firstly, since they are extremely
bright, we can see them at even great distances and therefore even from
the distant past — a supernova that we see now and that exploded 8
billion light-years away actually exploded 8 billion years ago and its
light is just reaching us now (one light-year is the distance light
travels in one year). Thus, supernovae tell us what the universe was
like long ago. Secondly, because type 1a supernovae always occur in the
same way, they all explode with the same amount of energy, emitting the
same amount of light. This allows us to measure how far away they
are/were because light intensity decreases in a well-known way as it
expands throughout space. Astronomers describe this by saying that type
1a supernovae are outstanding “standard candles” (a term that reflects
how old the science of astronomy is). And thirdly, type 1a supernovae
have distinctive signatures that allow astronomers to reliably
distinguish them from other cosmic explosions.
The origin of type 1a supernovae is
quite special and very interesting. Our Sun is a bit unusual in its
solitude — it has no companion stars — enabling its planets, including
Earth, to have stable orbits, vital for life. However, most stars have
nearby partners, and two-star systems, called “binaries”, are common.
Stellar partners can have quite different masses and heavier stars
evolve more rapidly. In a binary system with two ordinary stars (not
super-massive), the heavier star will reach the end of its life first,
becoming a red giant and then a white dwarf. White dwarfs are collapsed
stellar cores about the size of Earth with masses up to 140% of our
Sun’s. Their surface gravity can be one million times greater than
Earth’s. When its lighter companion becomes a red giant, a white dwarf
can capture gas from the red giant’s loosely held outer layers. The
white dwarf grows more massive at its partner's expense, in a form of
stellar cannibalism. When the white dwarf grows to 140% of our Sun’s
mass, a cataclysmic explosion utterly destroys the star. These
explosions all have the same origin and all explode at the same mass,
making type 1a supernovae superb “standard candles.”
The new Nobel Laureates used smaller
telescopes, which are more readily available, to repeatedly image
specific areas of the sky. Supernovae stood out as extremely bright
spots that appeared suddenly. They then used larger and more expensive
telescopes, including Hubble and Keck, to make higher precision
measurements of the supernovae’s intensity, spectrum, and redshift. Type
1a’s are distinguished by their spectrum and by how their intensity
varies over time.
Their peak intensity observed on Earth
determines how far away the supernovae are, and their redshift helps
determine the universe’s expansion rate at the time of the explosion.
That both groups independently came to
the same shocking conclusion made their results much more convincing.
Anyone can occasionally make a mistake, but it is far less likely that
two groups make exactly the same mistake.
So what does it all mean? What is this mysterious dark energy?
While we have ideas and some important
observations, the bottom line is that understanding dark energy is
cutting-edge science, or perhaps better described as bleeding-edge
science. Analysis of the cosmic microwave background radiation (CMB)
shows that 73% of all the energy in our observable universe is now in
the form of dark energy — it’s the vast majority of what’s out there.
Our best idea comes from a combination
of Quantum Mechanics and Einstein’s Theory of General Relativity.
Quantum Mechanics says that empty space is never truly empty. Due to
Heisenberg’s Uncertainty Principle, neither man nor nature can detect
very small energy changes that persist for very short time periods — the
smaller the energy change, the longer it can persist. And as Murray
Gell-Mann, one of my Caltech professors, famously said, “Whatever isn’t
forbidden is mandatory.” Thus, “virtual” particles can spontaneously
appear anywhere, anytime, and quickly disappear back into nothingness.
They borrow energy from the Bank Of Heisenberg on a very short-term
loan.
While these virtual particles “exist”,
they must have positive energy, however miniscule and fleeting. Since
they occur everywhere and always, they give even “empty” space some
amount of positive energy. And to conserve energy, virtual particles
must give space negative pressure. (The gas law, P dV = –dE, says that
as space expands, dV>0, and the amount of dark energy increases,
dE>0, the pressure, P, must be negative). General Relativity says
that an entity with energy and pressure exerts a gravitational
attraction proportional to E+3P, which in this case is negative. This
means virtual particles give space negative gravity, gravity that pushes
things apart rather than pulling things together as normal gravity
does. Several effects of virtual particles are precisely confirmed by
lab experiments.
If all this is indeed true, Perlmutter,
Schmidt, and Riess have shown us a brave new world. When the universe
was young and much smaller, there was less space and less dark energy.
The mutual gravitational attraction of the galaxies dominated, and the
expansion gradually decreased. As the universe became larger, there was
more space and thus more dark energy. Also, the mutual attraction of the
galaxies diminished as their separations expanded. At about 6 billion
years ago, the balance of power shifted; the repulsive gravity of dark
energy began to exceed the attractive gravity of matter (including dark
matter), causing the expansion rate to increase. As the universe
continues growing, dark energy becomes ever more dominant, and the
universe will accelerate ever faster.
That is the large-scale picture for the
universe. Galaxy clusters will move apart from one another ever faster.
But the clusters themselves will not expand. On this smaller scale, the
mutual gravity of all the massive objects in a cluster still dominates
its dark energy. If the cluster doesn’t expand, the amount of its space
and dark energy will not increase, and thus the balance will not shift.
Smaller structures will also not expand — our galaxy will not expand,
Earth will not expand, and we will not expand (except at Thanksgiving).
Best Regards,
Robert
October 2011
|